NUCPY unveils nuclear changes during glia-to-neuron reprogramming
Gabriel E Herrera-Oropeza, 2nd year PhD, King’s College London
BACKGROUND:
​
Direct lineage reprogramming consists of the conversion of one specialised cell type to another without going through an intermediate pluripotent stage. The strategy behind cell reprogramming is based on the knowledge of transcriptional networks that regulate the acquisition and maintenance of cell identity. Overexpressing specific transcription factors in a fully differentiated cell forces a rearrangement of its transcriptional network imposing a new molecular programme and therefore a new cellular identity. These newly converted cells have the potential to replace cells that were lost to disease or injury. This strategy is particularly attractive for the mammalian brain, where the lack of adult neuronal regeneration is a big hurdle for its natural repair. Due to their shared origin with neurons, proliferative capacity, and abundance in the brain, the conversion of glial cells into functional neurons represents a promising strategy for brain repair.
​
The nucleus is not a passive passenger within the cell. In the nervous system, nuclear architecture varies across cell types and throughout different stages of cell differentiation and development, impacting chromatin organisation and compaction, which is key for the regulation of gene expression and cell fate control. However, no study to date has explored the behaviour of nuclear morphology and chromatin compaction during glia-to-neuron reprogramming.
​
​
METHODOLOGY:
​
We transduced mouse cortical astroglia in vitro with a retroviral vector encoding neurogenic transcription factor Neurogenin2 and fixed cells every 24h from 1 to 6 days postinfection (DPI). We stained nuclei with DAPI and incubated the samples with anti-RFP (transduction reporter) and anti-βIII-tubulin antibodies to subsequently capture digital images with epifluorescence microscopy. Next, we developed a high content image analysis framework, Nucpy, that measuring morphological and structural features of the nuclei and combined with immunocytochemistry can infer cellular trajectories.
​
RESULTS:
​
We employed Nucpy to segment images of the nuclei and measure their features. We identified a total of 1589 cells (1 DPI: 131, 2 DPI: 126, 3 DPI: 231, 4 DPI: 373, 5 DPI: 510, 6 DPI: 218 cells) with high RFP intensity, which were most likely retrovirally transduced, and used them for the analysis here mentioned. By generating masks of the cell bodies and measuring their area, we found a positive correlation between nuclear area and cell body size, which tend to decrease during the reprogramming course (Figure 1a,b). We then used the average DAPI intensity of astrocytes at 1 DPI as a reference to estimate chromatin compaction and found that it negatively correlates with the nuclear area. Accordingly, as the nuclei shrink during neuronal conversion chromatin compaction intensifies (Figure 1c).
​
​
​
​
​
​
​
​
​
​
​
​
Figure 1. Nuclear size is reduced during neuronal conversion. (a) Correlation between nuclear area and cell body size from the 1st to 6th DPI. Each dot represents an individual cell (colours correspond to DPI), and boxplots represent the distribution of the data per day. (b) Typical nuclei (DAPI) found per DPI showing reduction in nuclear size. (c) Correlation between nuclear area and chromatin compaction per DPI. Each dot represents an individual cell.
​
Although comparing nuclear features between different DPI can give us an idea of their behaviour throughout time, cell reprogramming is more of an asynchronous process. To this end, we created a framework within Nucpy for organising cells in pseudotime according to their morphological characteristics and RFP and βIII-tubulin levels (Figure 2a). Using this approach, we identified three different nuclei populations: glial, intermediate stage, and iN nuclei (clusters 1, 2, and 3, respectively) (Figure 2b). Interestingly, we observed major changes in nuclear morphology and chromatin compaction during the intermediate stage (cluster 2) (Figure 2c), which is mainly integrated by cells from 4 and 5 DPI (Figure 2d).
​
​
​
​
​
​
​
​
​
​
​
​
​
​
​
​
​
​
​
​
​
​
​
​
​
Figure 2. Reconstruction of glia-to-neuron reprogramming trajectory using nuclear features. (a) Predicted glia-to-neuron trajectory shown in diffusion map. (b) Leiden clustering of transduced cells (RFP high). (c) Ordered changes in nuclear features according to the predicted trajectory. (d) Distribution of cells according to their DPI across clusters.
Altogether, this work describes changes occurring at the nuclear structure level during glia-to-neuron conversion aiming to help better understand its underlying mechanisms.
​
​
FUTURE WORK:
​
These findings shed light on possible mechanisms underlying in vitro glia-to-neuron reprogramming. However, further analyses and experimental validations are still required to better understand the implication of nuclear morphology and chromatin compaction on neuronal conversion.
The next step would be to morphologically characterise the nuclei of endogenous neurons and compare them to those of iNs. Only this would allow us to determine whether the nuclear changes here described are exclusive of an induced fate conversion process or if they are typical of a neuronal identity.
​
FUNDED BY:
​
​
​
​
​
​
​
CONTACT:
The Wellcome Trust
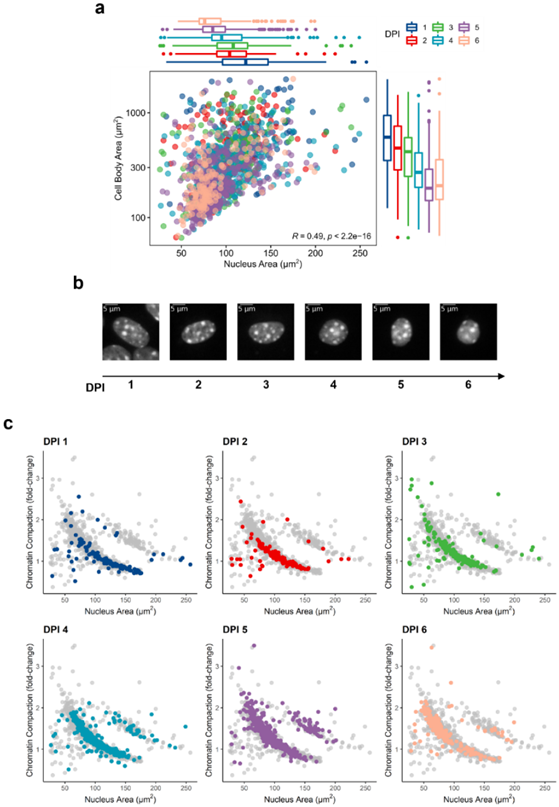

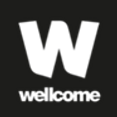
Gabriel Emilio Herrera Oropeza
Gabriel Emilio Herrera Oropeza